- Academic Editor
†These authors contributed equally.
Sepsis is defined as “a life-threatening organ dysfunction caused by a dysregulated host response to infection”. Although the treatment of sepsis has evolved rapidly in the last few years, the morbidity and mortality of sepsis in clinical treatment are still climbing. Sirtuins (SIRTs) are a highly conserved family of histone deacetylation involved in energy metabolism. There are many mechanisms of sepsis-induced myocardial damage, and more and more evidence show that SIRTs play a vital role in the occurrence and development of sepsis-induced myocardial damage, including the regulation of sepsis inflammation, oxidative stress and metabolic signals. This review describes our understanding of the molecular mechanisms and pathophysiology of sepsis-induced myocardial damage, with a focus on disrupted SIRTs regulation. In addition, this review also describes the research status of related therapeutic drugs, so as to provide reference for the treatment of sepsis.
Sepsis is a life-threatening disease that involves multiple organs of the human body. It is an important cause of global health burden due to its high mortality, long hospital stays and high cost. In recent years, many people have explored the pathophysiology and mechanism of sepsis, involved the interaction of multiple factors, such as the immune system, coagulation cascade, gut microbiota, neuroendocrine system, energy metabolism, and so on, which has led to different degrees of understanding of sepsis [1, 2]. However, the overall situation of sepsis is still uncontrollable. In addition, sepsis survivors have an increased risk of death or decreased health-related quality of life even after hospital discharge [3]. Therefore, sepsis is still one of the most important problems we need to solve. Studies have shown that sepsis inhibits energy metabolism of the cardiac, reduces ATP production, and causes myocardial damage [4]. Myocardial damage is one of the main complications of sepsis, and cardiac dysfunction can predict a poor clinical prognosis.
Sirtuins (SIRTs) are histone deacetylases III, divided into groups I to IV
(SIRT1-3, SIRT4, SIRT5, and SIRT6/7). SIRTs regulates a variety of physiological
functions ranging from energy metabolism to stress response and also exhibits
significant antioxidant activity, mainly due to its deacetylation and activation
of antioxidant enzymes [5]. A growing number of evidences support the critical
role of the highly conserved nicotinamide adenine dinucleotide
(NAD
Inflammation, oxidative stress, autophagy, and cardiomyocyte apoptosis ultimately contribute to cardiac dysfunction in sepsis [8, 9]. Large amounts of reactive oxygen species (ROS) and reactive nitrogen species are accumulated. When the oxidation/antioxidation becomes unbalanced, excessive ROS production can cause damage to the cardiac, as well as damage the structure of cardiomyocytes and promote apoptosis directly [10]. In addition, excessive ROS production during sepsis can exacerbate myocardial inflammation, attenuates the endothelium-dependent vasodilatory response and exacerbates microcirculatory disturbances [11].
During the acute infection stage of sepsis, some monocytes are activated and
produce a variety of cytokines such as tumor necrosis factor-
Several studies have reported that aberrant host responses mediated by Toll-like
receptors (TLR) contribute to the pathogenesis of sepsis [17, 18, 19]. TLR is
expressed in immune and other cells, including cardiomyocytes, and interacts with
different pathogen-associated molecular patterns (PAMPs), leading to activation
of NF-
Activation of the TLR4 signaling pathway may directly contribute to
cardiomyocyte dysfunction. Besides, sepsis directly impairs cardiac function by
binding to its receptor TLR4 through its binding protein CD14, which produces
inflammatory factors such as TNF-
MiR-146a is typically considered a negative regulator of the TLR4 and
NF-
Sepsis triggers endoplasmic reticulum stress, leading to activation of
calmodulin-dependent protein kinase kinase beta (CaMKK
Mitochondria, as energy suppliers to cardiomyocytes, contribute to the pathophysiology of myocardial dysfunction in sepsis [35]. Mitochondrial dysfunction associated with septic cardiomyopathy consists of the following: changes in mitochondrial structure (swelling, internal vesicle formation, and cristae abnormalities), mitochondrial DNA damage, elevated mitochondrial permeability transition, and inhibition of cytochrome C oxidase activity [36, 37, 38, 39]. Sepsis causes mitochondrial damage through multiple mechanisms [40, 41]. Damaged mitochondria promote cardiomyocyte apoptosis, which in turn exacerbates sepsis-induced myocardial damage. Biogenesis of damaged or defective mitochondria is associated with accumulation of useless or dysfunctional mitochondria with reduced mitochondrial potential or increased ROS [42]. These damaged mitochondria subsequently interfere with cardiomyocyte metabolism and induce mitochondrial apoptosis or necrosis via activation of 1-Methyl-4-phenyl-1,2,3,6-tetrahydropyridine (mPTP) [43, 44].
Mitochondrial damage and dysfunction are highly associated with sepsis-induced myocardial damage [45, 46]. Mitochondria are the main source of intracellular ROS in the myocardium during sepsis. Sepsis damages myocardial mitochondria by disrupting membrane integrity, which in turn increases ROS production and oxidative stress [47]. Increased ROS production leads to oxidative stress that impairs mitochondrial biosynthesis, division/fusion homeostasis, and mitochondrial autophagy, thereby promoting apoptosis, hypertrophy, and remodeling, which have been widely reported in sepsis-induced cardiac, renal, and pulmonary damage [48]. The expression of Gasdermin D (GSDMD) functional fragment is up-regulated in the heart tissue of septic WT mice, accompanied by reduced cardiac function and myocardial injury. GSDMD leads to mitochondrial dysfunction and ROS overproduction by enriching mitochondria, which in turn regulates the activation of NLRP3 inflammasome, leading to myocardial injury, pyroptosis, and cell death in sepsis. GSDMD enrichment in the mitochondrial membrane triggers ROS production and may be partially involved in NLRP3 activation [49, 50]. NLPR3-deficient mice show improved cardiac systolic and diastolic function and increased survival from sepsis [51]. GSDMD plays a role in sepsis plays an important role in the pathophysiology of sepsis-induced myocardial damage [50].
In addition, inhibition of the NLRP3/IL-1
We summarized the current mechanism of myocardial damage caused by sepsis, and then we started from the damage mechanism to explore whether SIRTs can inhibit these damages and thus achieve protective effects. The molecular mechanisms of mitochondria associated with myocardial damage caused by sepsis are summarized in Fig. 1.
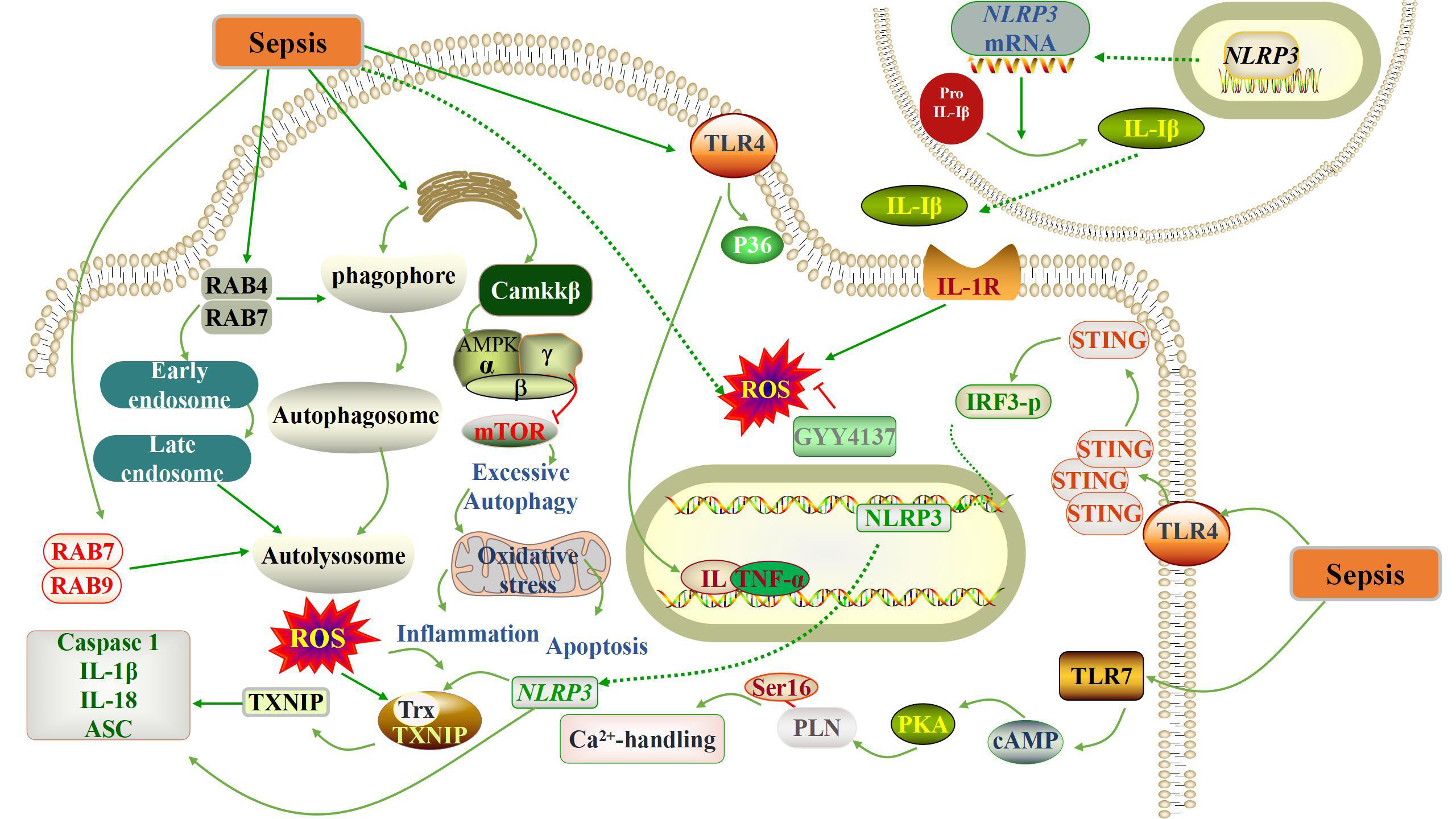
Schematic representation of the pathogenesis of sepsis-induced
myocardial damage. Sepsis triggers a complex cascade of reactions, including the
activation of oxidative stress, the occurrence of autophagy, apoptosis and
systemic inflammatory response. IL, interleukin; AMPK, adenosine 5
SIRT1 is a conserved NAD
Clinical studies have found that SIRT1 can still be clinically relevant as a
rapid measurable prognostic biomarker for patients with sepsis in the clinical
setting [53]. SIRT1 expression is downregulated in the hyper-inflammatory
response to sepsis, and SIRT1 activation counteracts sepsis-induced myocardial
damage [54, 55, 56]. Besides, SIRT1 played a negative role in regulating the
activation of NLRP3 inflammasome [57, 58]. NLRP3 interacts with
apoptosis-associated speckle-like protein (ASC) and promotes its own cleavage and
activation through ASC recruitment of caspase1 precursor monomers. Thus,
activated caspase 1 stimulates the cleavage of pro-IL-1
Besides, injection of a cell-permeable Tat-Beclin-1 peptide to activate
autophagy improved cardiac function, attenuated inflammation, and rescued the
phenotypes caused by Beclin-1 deficiency in sepsis-challenged mice, this suggests
that Beclin-1 protects against cardiac damage during sepsis [62]. miR-181a is one
of the MicroRNAs that represses gene expression at the post-transcriptional level
by binding to target mRNAs [63]. MiR-181 levels were significantly increased in
the presence of enhanced inflammatory response and apoptosis. MiR-181 binds
directly to its target genes and inhibits SIRT1 expression. MiR-181a inhibition
leads to upregulation of SIRT1 expression and downregulation of pro-inflammatory
cytokines and apoptosis. Inhibition of miR-181a attenuates sepsis-induced
inflammation and apoptosis by activating the Nuclear factor erythroid2-related
factor 2 (Nrf2) pathway while inhibiting the NF-
Restoring mitochondrial quality control mechanisms by activating mitochondrial
biosynthesis or reducing mitochondrial division is a promising approach to
improve organ dysfunction in sepsis [69, 70]. SIRT1/peroxisome
proliferator-activated receptor-gamma co-activator-1alpha (PGC-1
SIRT2, far less studied than SIRT1, is mainly located in the cytoplasm, but
under certain conditions, it also enters the nucleus and performs the
corresponding functions [74]. SIRT2 is a nutrient sensor in adipose and immune
cells, like SIRT1, increased caloric restriction lead to a high nutrient and high
fat diet decreases SIRT2 expression in white adipose tissue of mice [75, 76]. In
obese mice with sepsis, elevated levels of SIRT2 protein instead prolonged low
inflammation; SIRT2 is the most abundant SIRTs in adipose tissue [77].
Specifically, there are some studies found that through direct deacetylation of
NF-
SIRT3 is an NAD
Mitochondrial dysfunction impairs the contractility of cardiomyocytes and even
triggers cardiomyocyte death [90]. Annexin A1 (ANXA1), a glucocorticoid-regulated
protein that is widely expressed in human tissues and cells [91]. ANXA1 promotes
p53 deacetylation in sepsis-treated H9C2 cells by enhancing SIRT3 expression
thereby reducing cardiomyocyte death [92]. Impaired SIRT3 activity may mediate
cardiac dysfunction in endotoxemia by promoting calpain-mediated disruption of
ATP synthesis, suggesting SIRT3 activation as a potential therapeutic strategy
for the treatment of septic cardiomyopathy [87]. Tubeimoside I (TBM) prevents
sepsis-induced endothelial dysfunction by reducing oxidative stress and apoptosis
through SIRT3. TBM is a promising new therapeutic agent for sepsis-induced
endothelial dysfunction [89]. Polydatin-mediated SIRT3 activation preserves
mitochondrial function through mitochondrial superoxide dismutase 2 (SOD2) and
Cyclophilin D (CypD) deacetylation, thereby reversing sepsis-induced endothelial
hyperpermeability [93]. In addition, the role of SIRT3-CypD signaling in barrier
protection was identified. SIRT 3-mediated deacetylation inhibited CypD activity,
thereby inhibiting mPTP opening and
SIRT3 deficiency leads to hyperacetylation of key enzymes in the cardiac trichloroacetic acid (TCA) cycle, which subsequently shifts myocardial metabolism to anaerobic glycolysis and subsequently promotes lactate and nicotinamide adenine dinucleotide (NADH) production, ultimately exacerbating cardiac function after sepsis [88]. SIRT3 overexpression increases AMPK activity and improves mitochondrial biosynthesis, which maintains mitochondrial function and reduces sepsis-associated cardiomyocyte damage [90]. These studies suggest that targeting SIRT3 may provide a potential new target to maintain normal cardiac performance after sepsis. However, the precise mechanisms of protection still need further investigation.
Unlike SIRT1 and SIRT3 deacetylases, SIRT5 has relatively weak deacetylation,
but shows stronger desuccinylation activity, mainly removing succinyl, malonyl
and glutaryl groups from lysine residues of mitochondria and peroxisome metabolic
enzymes [96]. For example, SIRT5 has been reported to be able to descuccinylate
pyruvate dehydrogenase 1 (PDHA1) and succinate dehydrogenase (SHDB), and play a
role in regulating the activity of these protein [97]. As a member of SIRTs,
SIRT5 has been proved to be located in mitochondria and cytosol [98]. As far as
its cell biological function is concerned, SIRT5 can promote ammonia
detoxification, regulate glycolysis, TCA cycle and electron transfer chain. In
addition, SIRT5 can also promote fatty acid
In sepsis, SIRT5 has also attracted great attention. Studies have shown that in
sepsis, SIRT5 can enhance the innate inflammatory response of macrophages or
endotoxin-tolerant macrophages by promoting the acetylation of p65 and activating
NF-
SIRT6 is another protein that depends on NAD
As far as sepsis is concerned, SIRT6 can reduce the inflammatory reaction of human venous endothelial cells (HUVECs) induced by sepsis by positively regulating the expression of Nrf2 and activating anti-inflammatory and antioxidant enzymes regulated by Nrf2, which suggests that SIRT6 is a possible target for preventing lung damage induced by sepsis [105]. Further research shows that the overexpression of SIRT6 in renal proximal convoluted tubule epithelial cells will alleviate SAKI by activating autophagy, which promotes AMPK activation, inhibits mTOR signaling pathway, promotes the polarization of macrophage M2 in autophagy-dependent and non-autophagy-dependent ways, and then plays a role in alleviating SAKI [104]. In another experiment of damage and apoptosis of renal tubular epithelial cells induced by sepsis, it was found that SIRT6 can activate nuclear factor erythroid-2-related factor 2-antioxidant response element (Nrf2)/ARE signal, alleviate renal dysfunction induced by sepsis, and reduce apoptosis and oxidative stress of renal tubular epithelial cells [106].
Because SIRT6 can promote autophagy, studies show that the overexpression of SIRT6 can alleviate sepsis-induced apoptosis, and further induce autophagy to play a protective role in renal epithelial cell damage caused by sepsis [107]. On the one hand, the up-regulated expression of SIRT6 can promote autophagy, on the other hand, it can protect renal tubular epithelial cells from oxidative stress, inflammatory reaction and apoptosis, and then play a protective role in sepsis-induced renal damage [108]. Another study on the treatment of sepsis shows that the activation of SIRT6 can inhibit the apoptosis of myocardial cells and the expression of inflammatory factors by promoting autophagy, and then inhibit the myocardial dysfunction induced by sepsis [109]. Besides promoting autophagy, SIRT6 plays an important role in regulating glucose metabolism in vivo, and the decrease of SIRT6 activity will reverse glycolysis block related to monocytes caused by endotoxin, which is a process that leads to immune metabolism paralysis of monocytes in human and mice sepsis [110]. This shows that SIRT6 mainly promotes autophagy, reduces oxidative stress and alleviates sepsis mediated by inflammatory factors.
SIRT7 is also a deacetylase belonging to SIRT family. Many studies have shown that SIRT7 can regulate protein deposition, endoplasmic reticulum stress, mitochondrial protein folding and mitochondrial metabolism [111]. SIRT7 is mainly located in nucleoli [112], which combines with ribosomal RNA gene and participates in the transcription process of ribosomal DNA during mitosis. One of its important functions is to regulate chromatin remodeling [113]. SIRT7 can exert deacetylation activity at DNA damage sites, and show other catalytic activities for protein involved in DNA damage and repair [111, 112]. Although there are few studies on the role of SIRT7 in sepsis at present, a study on the mechanism of resveratrol found that the activation of SIRT7 can reduce inflammatory factors and play a positive role in the treatment and protection of sepsis and its cardiac damage [114]. Another study shows that liver damage will be caused during the pathogenesis of sepsis, and the activation of endoplasmic reticulum stress (ERS) pathway will cause apoptosis of hepatocytes. SIRT7 can inhibit glucose-r\regulated protein 78 (GRP78) in ERS pathway to inhibit apoptosis of hepatocytes, which provides clues for the treatment of liver damage caused by sepsis in the future [115]. Due to its unique biological function, SIRTs reduces sepsis by deacetylating several histone and non-histone proteins. Fig. 2 summarizes the role of these SIRTs in cells and their mechanisms of action in sepsis.
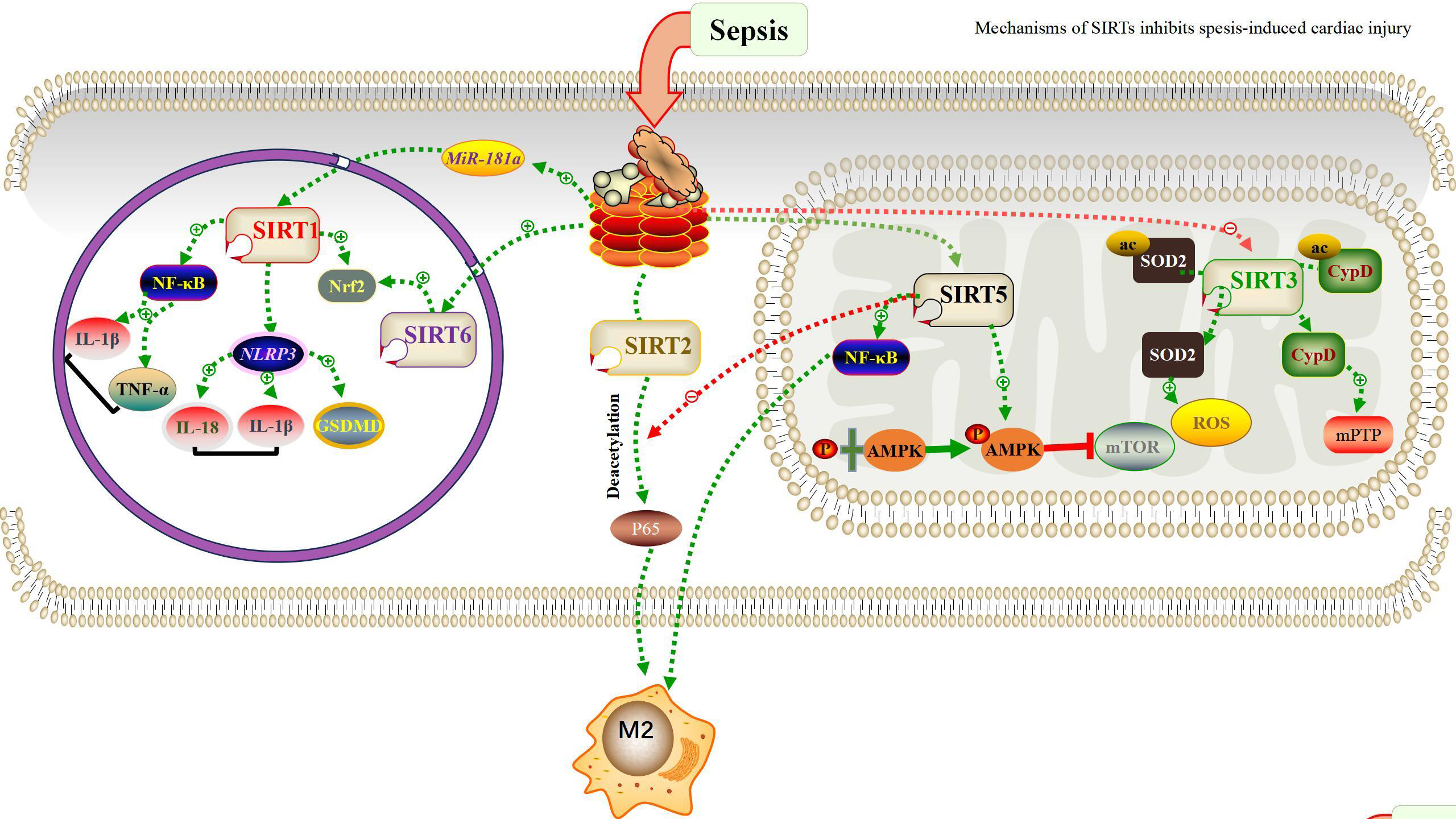
Schematic representation of SIRTs modulates the
inhibition of sepsis through intracellular mechanisms. SIRTs is dispersed in
subcellular compartments of cells. Each SIRTs has its own unique targets that
define its biological activity, as described in the review. SIRT, Sirtuin; NF-
As mentioned above, SIRTs play an important role in the development of sepsis.
Therefore, it is extremely important to find a cure for sepsis with SIRTs as the
target. On the one hand, the up-regulation of SIRT1 induces deacetylation of
NF-
In summary, SIRTs are involved in important metabolic reactions and play an important role in the development of sepsis. We have witnessed great advances in our understanding of sepsis in the SIRTs involved. However, our exploration of the upstream and downstream pathways of SIRTs is very limited, we will also focus on the SIRTs up-down pathway. We believe that it is feasible to regulate the occurrence and development of sepsis by regulating the level of SIRTs, and the subsequent research on its mechanism and the development of new drugs will open up new avenues for the treatment of sepsis.
ZP and WY: contributing to conceptualization ideas; formulation and evolution of review aims; contributing to management and coordination responsibility for the research activity planning and execution; revise the draft. SW and YW: contributing to the visualization preparation, creation and presentation of the published work; contributing to write the initial draft. All authors contributed to editorial changes in the manuscript. All authors read and approved the final manuscript. All authors have participated sufficiently in the work and agreed to be accountable for all aspects of the work.
Not applicable.
Not applicable.
This study was funded by Liaoning Province Applied Basic Research Program [grant number 2023JH2/101300098] and Beijing Jiekai Cardiovascular Health Foundation [grant number BW20220302].
The authors declare no conflict of interest. Zuowei Pei is serving as one of the Guest editors of this journal. Zuowei Pei had no involvement in the peer-review of this article and has no access to information regarding its peer review. Full responsibility for the editorial process for this article was delegated to Graham Pawelec.
Publisher’s Note: IMR Press stays neutral with regard to jurisdictional claims in published maps and institutional affiliations.