- Academic Editor
†These authors contributed equally.
Background: Hypoxic-ischaemic encephalopathy (HIE) is a major cause of
neonatal disability and mortality. Although hypothermia therapy offers some
neuroprotection, the recovery of neurological function is limited. Therefore, new
synergistic therapies are necessary to improve the prognosis. Mesenchymal stem
cell-based therapy is emerging as a promising treatment option for HIE. In this
study, we studied the therapeutic efficacy of human placenta-derived mesenchymal
stem cells (PD-MSCs) in the HIE rat model and analyzed the underlying therapeutic
mechanisms. Methods: Rats were divided into 6 groups (n = 9 for each) as
follows: control, HIE model, HIE + normal saline, and HIE + PD-MSC
transplantation at days 7, 14 and 28 postpartum. Following PD-MSC
transplantation, neurological behavior was evaluated using rotarod tests,
traction tests, and the Morris water maze test. The degree of brain tissue damage
was assessed by histological examination and Nissl staining. Expression levels of
apoptosis-related proteins and inflammatory factors were quantified by Western
blotting and enzyme-linked immunosorbent assays. Immunofluorescence was used to
investigate the ability of PD-MSCs to repair the morphology and function of
hippocampal neurons with hypoxic-ischaemic (HI) injury. Results: PD-MSC
transplantation enhanced motor coordination and muscle strength in HIE rats. This
treatment also improved spatial memory ability by repairing pathological damage
and preventing the loss of neurons in the cerebral cortex. The most effective
treatment was observed in the HIE + PD-MSC transplantation at day 7 group.
Expression levels of microtubule-associated protein-2 (MAP-2), B-cell lymphoma-2
(BCL-2), interleukin (IL)-10, and transforming growth factor (TGF -
Perinatal hypoxia-ischaemia (HI) is a major cause of newborn disability and
mortality, especially hypoxic-ischaemic encephalopathy (HIE), which is the
leading cause of cerebral palsy in neonates [1]. The worldwide incidence of HIE
is approximately 5/1000 live births. Approximately 20–25% of affected
individuals die during the neonatal period, and 25% of the survivors suffer
lifelong neurological sequelae [2]. The major causes of HIE are intrapartum HI
events, such as uterine rupture, placental abruption, cord prolapse, and amniotic
fluid embolism [3]. The pathogenesis of HIE injury may be related to
inflammation, oxidative stress and neuronal apoptosis, which are secondary to
oxygen and blood shortage [4]. Hypothermia is the standard treatment for neonates
with HIE and can reduce the risk of death and major neurodevelopmental
disabilities [5]. Although therapeutic hypothermia has become the standard
treatment for babies with moderate to severe HIE, the mortality rate after this
treatment is still as high as 27%, and almost 28% of surviving babies develop
major neurodevelopmental disabilities [2]. However, some patients cannot receive
hypothermia treatment if the gestational age is
Preclinical studies have confirmed that mesenchymal stem cells (MSCs) may have neurotherapeutic effects, and MSC-based therapy is emerging as a promising treatment option for HIE [7]. MSCs are undifferentiated, pluripotent stem cells with self-renewal properties and immunomodulatory capacity. These cells have been investigated as a potential treatment for HIE, as they reduce inflammatory and reactive oxygen species (ROS) damage, and promote the restoration of tissue function [7]. The mechanism by which MSC transplantation improves central injury could involve mitochondrial transfer, modulation of the immune-inflammatory response, and regulation of ROS production [7]. MSCs originate from the bone marrow, peripheral blood, muscle, adipose tissue, umbilical cord, placenta, etc. Placenta-derived mesenchymal stem cells (PD-MSCs) are an ideal source for such treatment, as they are easy to access and have self-renewal capabilities. Compared to bone marrow-derived MSCs (BM-MSCs), PD-MSCs have high proliferative activity and are strongly immunosuppressed [8]. Moreover, BM-MSCs are difficult to obtain due to the very invasive nature of bone marrow collection. The characteristics of adipose-derived MSCs (AD-MSCs) can vary considerably between patients according to biological factors such as age, sex, body mass index and disease [9]. Although some methods have been described for maintaining the stemness and proliferative potential of AD-MSCs, there are still some limitations with their potential clinical application [10]. The neuroprotective effect of MSCs has been observed in postnatal rat models created by blocking the unilateral carotid arteries, followed by exposure to a hypoxic environment [11, 12, 13]. However, this model does not precisely match the clinical conditions of HIE, which is often due to poor intrauterine blood perfusion and oxygen supplementation during the peripartum period. To investigate the neuroprotective effect of MSCs during the acute phase of HIE, MSC transplantation was performed within 6 hours after HI. Other research that focused on the secondary phase (lasting hours to days after HI) mostly used a transplantation time of between 1 to 10 days post-insult [14]. Few studies to date have focused on the tertiary phase, lasting from days to years after HI. Since HIE involves progressive neuronal cell loss over a period of days and months after HI, the neuro-restorative effect of MSC transplantation is worthy of further investigation. In the present study we developed an intrauterine HI rat model to investigate the neuroprotective effect and optimal treatment timing of PD-MSCs in neonatal rats with HIE. This new model was based on the rabbit intrauterine HI model of cerebral palsy [15, 16].
All experiments on human tissues were approved by the Ethics Committee of the
Zhejiang Provincial People’s Hospital, Zhejiang, China.
Written informed consent was obtained from all
subjects. PD-MSCs were isolated from placental villous tissues procured from
healthy women during labor. Briefly, placental villous tissues were cut into
approximately 1 mm
PD-MSCs were characterized by their morphology, surface marker expression, and mesenchymal lineage differentiation, as previously described [17]. Osteogenic, adipogenic and chondrogenic inductions were performed to evaluate the multi-lineage differentiation capacity of PD-MSCs. To evaluate mineralization, the cultures were stained with Alizarin Red S according to the manufacturer’s instructions (HUXXC-90021, Cyagen Biosciences Inc, Santa Clara, CA, USA). Briefly, cells were fixed with 4% paraformaldehyde (P0099, Beyotime Institute of Biotechnology, Shanghai, China) for 30 minutes and then stained with Alizarin Red S for 5 minutes at room temperature. The lipid droplets formed following induction of adipogenesis were visualized using Oil Red O staining (HUXXC-90031, Cyagen Biosciences Inc). Successful chondrogenic differentiation was tested by Alcian blue staining (HUXXC-90041, Cyagen Biosciences Inc).
The PD-MSC phenotype after the third passage was analysed by flow cytometry (BD FACSVerse, BD Biosciences, Franklin Lakes, NJ, USA) using the following antibodies: anti-CD14 (BD Biosciences, Franklin Lakes, NJ, USA, cat. no. 555397, 1:5 dilution), anti-CD45 (BD Biosciences, cat. no. 560973, 1:5 dilution), anti-CD19 (BD Biosciences, cat. no. 563326, 1:5 dilution), anti-CD34 (BD Biosciences, cat. no. 555822, 1:5 dilution), anti-CD73 (BioLegend, San Diego, CA, USA, cat. no. 344004, 1:20 dilution), anti-CD105 (BioGems, Westlake Village, CA, USA, cat. no. 17111-60, 1:20 dilution), anti-HLA-DR (BD Biosciences, Cat. no. 555811, 1:5 dilution) and anti-CD90 (BioLegend, cat. no. 328110, 1:5 dilution). Incubation with each of these antibodies was at room temperature for 15 minutes according to the manufacturer’s instructions. A suitable isotype-matched antibody (PE; BD Biosciences, cat. no. 555749; FITC, BD Biosciences, cat. no. 555573, 1:5 dilution) incubated at room temperature for 15 minutes was utilized as a negative control. The data were analyzed using BD FACSuite software (version 1.0.5.3841, BD Biosciences, Franklin Lakes, NJ, USA).
All animal experiments were approved by the Institutional Animal Care and Use
Committee of Zhejiang Provincial People’s Hospital. Pregnant Sprague Dawley rats
(Shanghai SLAC Laboratory Animal Co. Ltd, Shanghai, China) at day 18 of gestation
were used to isolate hippocampal neurons from the brains of late rat embryos.
Isolation and culture of the hippocampal neurons was carried out using a
previously described protocol [18]. Briefly, rat embryos were harvested from
pregnant rats at 18 days gestation. They were then dissected under a
stereomicroscope and in an ice-cold and sterile environment. After exposing the
surface of the cerebral cortex, the hippocampus was gently separated, cut into 1
mm
To construct an in vitro hypoxic-reoxygenated cell model, hippocampal
neurons were grown for six days in normal culture (37
°C, 5% CO
Cell cultures were fixed with 4% paraformaldehyde and permeabilized with 0.5%
Triton X-100 (Sigma-Aldrich, St. Louis, MO, USA) for 10 minutes each, incubated
with 5% bovine serum albumin (BSA) for 1 hour, and then stained overnight at 4
°C with mouse anti-neuron-specific enolase (anti-NSE; ab218388, Abcam,
Waltham, MA, USA) or rabbit anti-growth-associated protein 43 (anti-GAP-43;
AF0150, Beyotime Institute of Biotechnology). Subsequently, the cells were washed
with PBS and incubated for 30 minutes at room temperature with Alexa Fluor
488/594-conjugated secondary antibodies (Jackson ImmunoResearch, West Grove, PA,
USA). After further washes with PBS and counterstaining with
4
The HIE model was established based on previous studies by clipping the
bilateral uterine arteries for 5 minutes and delaying caesarean delivery [15, 19]. Pregnant Sprague-Dawley rats (21 days gestation) underwent general
anaesthesia using 3% isoflurane in a 30% O
The newborn pups were randomly assigned to six groups (9 pups per group) as
follows: control group, HIE model group, HIE + normal saline (NS) group, and
three HIE + PD-MSC treatment groups (transplantation at days 7, 14 and 28
postpartum, giving the P7, P14 and P28 groups, respectively). Five microlitres of
PD-MSC cell suspension (5
Rats were evaluated for neurological function at 56–60 days postpartum.
The rotarod test was conducted to evaluate motor performance. The rats were placed on a rotarod apparatus (ZH-ZQ, Zhenghua, Huaibei, Anhui, China) with a starting speed of 0 rpm and accelerating 2 rpm every 5 seconds until reaching 45 rpm. The time that each rat spent on the rotary was recorded. Rats that remained on the apparatus after 60 seconds were given a score of 60 seconds.
The traction test was performed to evaluate muscle strength and equilibrium. The front paws of the rats were placed on a straight rope hung in the air. Scoring was determined as follows: if both paws held on, the score was 3 points; if one paw held on, the score was 2 points, and if neither paw held on the score was 0 points. The test was repeated three times, with a 3 min rest between trials. The average score was calculated for data analysis.
The Morris water maze (MWM) test was performed to evaluate the spatial learning and memory of rats. The rats were trained to find a platform in a large open circular tank filled with tap water (150 cm in diameter, 45 cm in height, and filled to a depth of 30 cm with water at 28 °C). A 10 cm diameter white platform was placed in the center of target quadrants of the pool. On day 1, the rats were trained to find the platform 0.5 cm above the water. Rats were then trained for four more days to find the submerged platform placed 0.5 cm below the surface of opaque water. Each rat performed four consecutive trials from a different quadrant each day, and with a 20-second interval between two trials. The rats were placed in the water between quadrants, facing the wall of the pool with a different location for each trial. If the rats failed to locate the platform within 90 seconds, they were gently guided onto the platform where they remained for 20 seconds. The platform position was kept unaltered during the training session. On day 6, the platform was removed, and each rat was allowed to explore the pool for 90 seconds. The mean escape latency time to locate the hidden platform in all four quadrants and the number of crossings of the platform were measured using an image analyzer (ZH0065, Zhenghua).
After neurological evaluation, five rats from each group were chosen randomly and the brains collected under anaesthesia. Brain tissues were dehydrated in ethanol and embedded in paraffin blocks. Five-micrometer-thick slices were dewaxed in xylene, rehydrated in progressively lower concentrations of ethanol, and then fully stained with haematoxylin and eosin (H&E) (C0105M, Beyotime Institute of Biotechnology) or cresyl violet (G1430, Solarbio).
At 60 days after HIE injury, brain tissue homogenates were prepared for Western blot analysis according to the manufacturer’s instructions. Briefly, brain tissue was added to a cold lysis buffer (P0013B, Beyotime Institute of Biotechnology), and the supernatants were collected and quantified using a BCA protein assay kit (P0011, Beyotime Institute of Biotechnology). Samples (20 µg) were separated on 10% polyacrylamide gels and transferred to Hybond-P membranes. Incubation of the membranes with a BSA solution (5%; ST025, Beyotime Institute of Biotechnology) at room temperature for 45 minutes was used to block non-specific binding. Primary antibodies against the following proteins were then added and incubated at room temperature for 45 minutes: B-cell lymphoma-2 (BCL-2; 12789-1-AP, Proteintech Group, Inc. Rosemont, IL, USA), BCL-2-associated X protein (BAX; AF0057, Beyotime Institute of Biotechnology), BCL-2-associated agonist of cell death (BAD; AF1009, Beyotime Institute of Biotechnology), neurofilament H/M (NF-H/M; 835403, BioLegend), microtubule-associated protein-2 (MAP-2; 822501, BioLegend), Caspase3 (AB3623, Sigma-Adlrich, St. Louis, MO, USA), and Caspase7 (AB256474, Abcam). GAPDH (AF1186, Beyotime Institute of Biotechnology) served as the loading control. The blots were then incubated with an HRP-conjugated goat anti-rabbit secondary antibody (A0208, Beyotime Institute of Biotechnology) for 45 minutes at room temperature, and subsequently analyzed using an enhanced chemiluminescence (ECL) kit (Beyotime Institute of Biotechnology). ImageJ (National Institutes of Health, Bethesda, MD, USA; http://rsb.info.nih.gov/ij/, v1.8.0) was employed to analyze the greyscale values.
ELISA kits were used to determine the concentrations of tumor necrosis factor
Data are presented as the mean and standard deviation (mean
PD-MSC were derived from placental villous tissues procured from healthy women during labor. The cells exhibited a bipolar spindle-like shape and displayed a high capacity to adhere to plastic when maintained in standard culture conditions using tissue culture flasks (Fig. 1A). PD-MSCs showed the capacity to differentiate into adipocytes, chondrocytes, and osteoblasts after being cultured in the appropriate induction conditions (Fig. 1B). Flow cytometry showed that PD-MSCs had positive expression of CD73 (99.8%), CD90 (99.4%) and CD105 (95.2%), and negative expression of the hematopoietic lineage markers CD14 (0.32%), CD19 (0.04%), CD34 (0.03%), CD45 (1.72%) and HLA-DR (0.02%). These differentiation abilities and the surface marker expression profile were in accordance with typical MSC standards (Fig. 1C).

Characteristics of human placenta-derived mesenchymal stem
cells. (A) Morphology of MSCs. (B) Differentiation of MSCs was observed after
induction (original magnification
We developed a new type of intrauterine HI rat model in order to study the
therapeutic efficacy of PD-MSCs and the underlying mechanism of action. Rats in
the HIE group had lower body weight than those in the control group. After
treatment with PD-MSCs, the mean weight was higher in the P7 (266.62
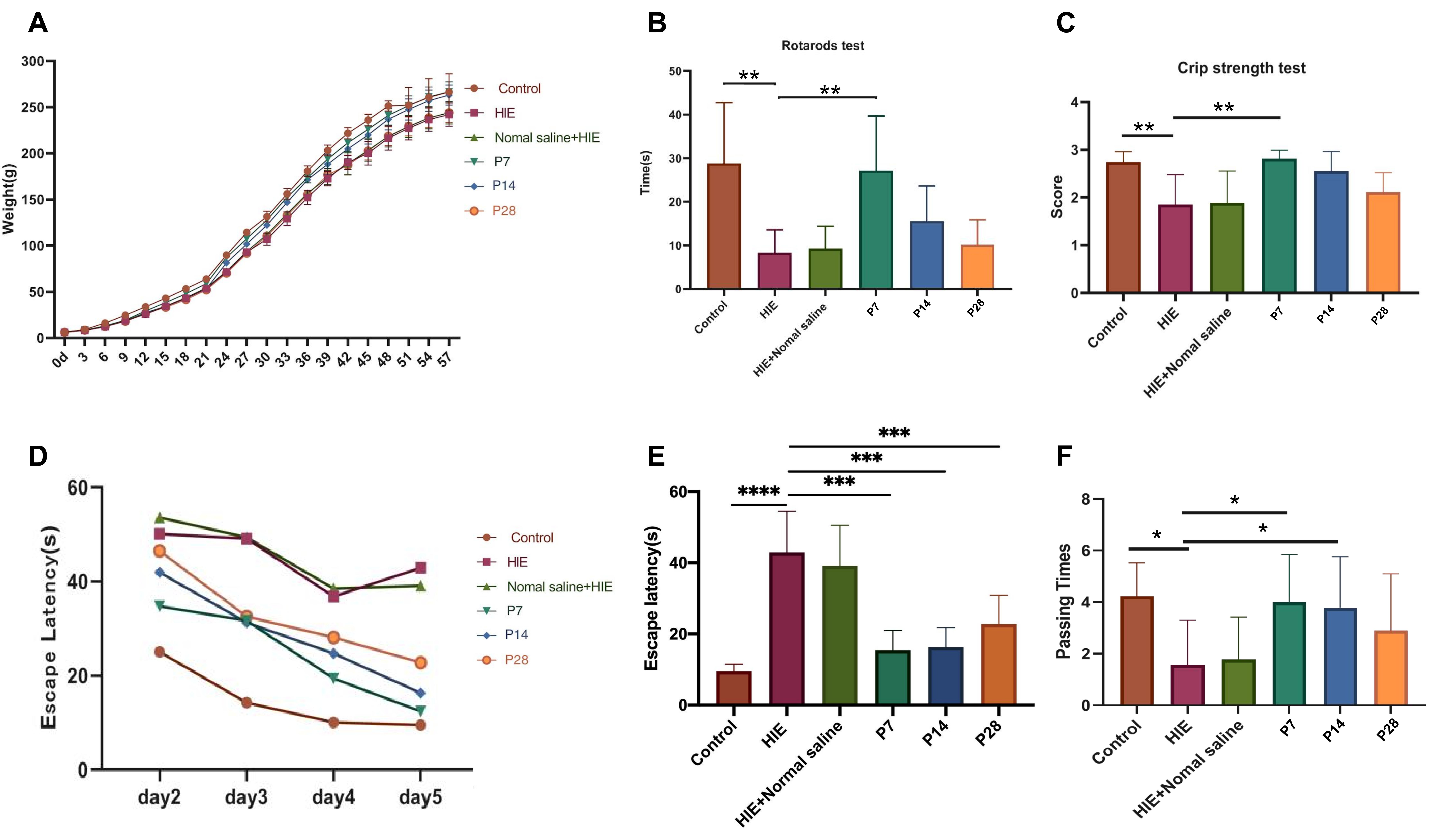
Body weight and neurological evaluation in the HIE
model. (A) Weight gain by the rats. At 56 days after PD-MSC injection, the mean
body weight increased until it was almost equal to the control group. (B) Rotarod
test. The significantly longer time spent on the rotarod indicated that PD-MSC
treatment improved coordination after HIE injury. (C) Traction test. Significant
improvement in muscle strength was observed after PD-MSC injection. (D) Escape
latencies for each group from the second to the fifth day of the test. The escape
latencies to locate the hidden platform gradually decreased in all three PD-MSC
treatment groups. (E) The escape latencies for each group on the fifth day. The
P7 group showed the best results compared to the P14 and P28 PD-MSC injection
times. (F) Passing times of probe trains. The significant reduction in time spent
locating the platform and the increased number of passages confirmed that PD-MSC
injection improved learning memory function after HIE injury. Data are mean
The neurological behaviors of motor coordination and balance in the HIE model
were assessed using the rotarod and traction tests. The rotarod test revealed a
prolonged mean latency time in the P7 (27.19
Morris water maze tests were performed to evaluate the capacity to recover
learning memory function in the HIE model. No significant differences in escape
latencies between each group were observed on the first day of the water maze
test, suggesting that neither HIE injury nor PD-MSC injection impaired rat
motility and vision. The test was then performed with the hidden platform from
the second to fifth days, with the escape time becoming progressively shorter
with increasing training time (Fig. 2D). Compared to the HIE group (42.90
Histological examination and Nissl staining were carried out to evaluate the degree of brain tissue damage in the HIE model. The H&E results revealed that PD-MSC transplantation reduced histopathological damage to the brain tissue caused by HIE (Fig. 3A). Furthermore, Nissl staining showed frequent neuronal degeneration and loss in the cerebral cortex after HIE injury (Fig. 3B). PD-MSC transplantation alleviated brain injury, and cell counts showed an increased number of neurons in the PD-MSC treatment groups (Fig. 3C). Western blot analysis showed a low level of MAP-2 expression in the HIE group. However, PD-MSC injection on the 7th day after HIE injury (P7 group) increased the level to close to the normal value (Fig. 3D). The P7 group had more neurons and greater MAP-2 expression than both the P14 and P28 groups, suggesting the optimal treatment time was approximately the 7th day after HIE injury.
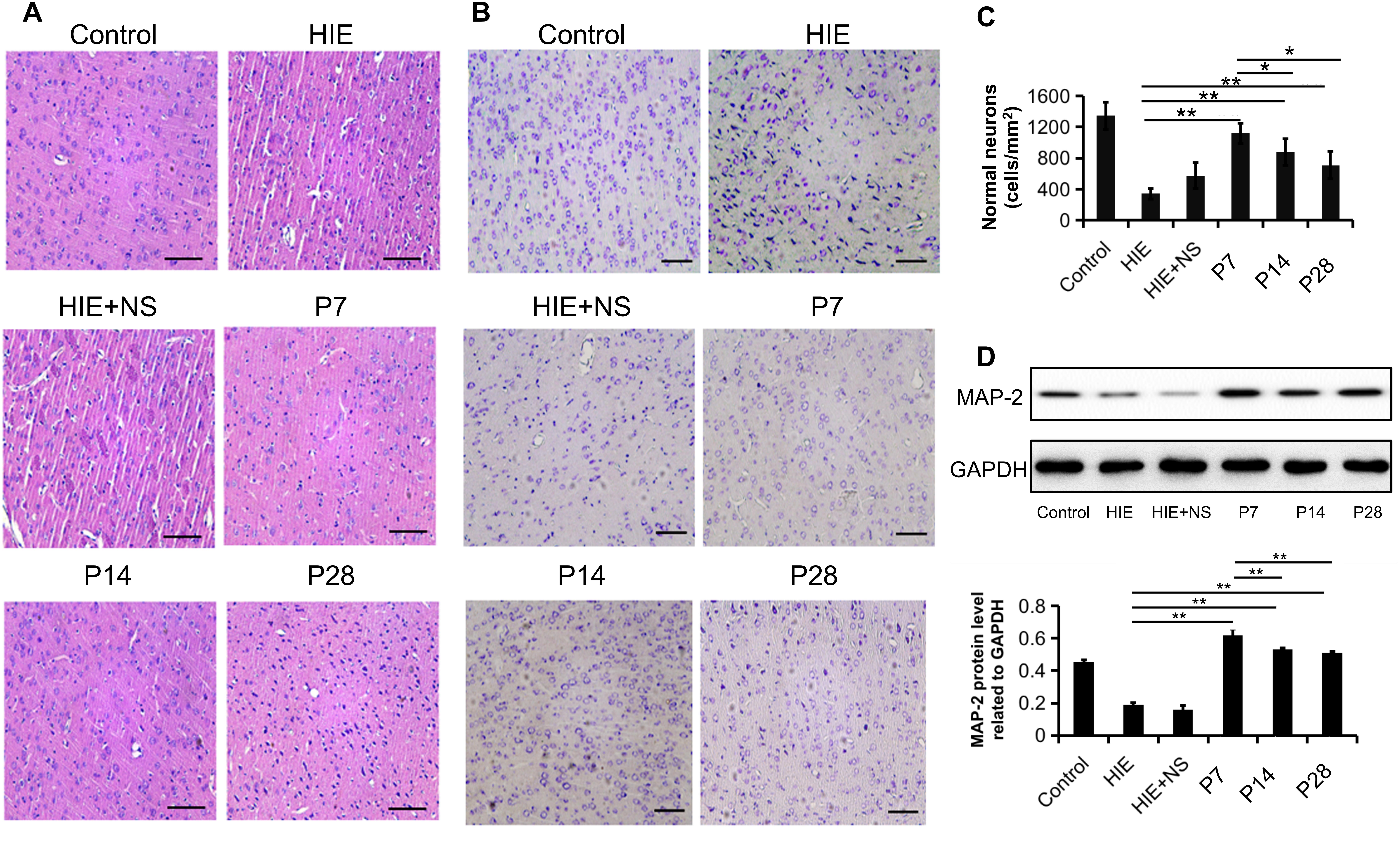
Histopathological results and neuron counts. (A) H&E
examination of histopathological damage in brain tissues. (B) Nissl staining was
used to assess neurons 60 days after HIE damage. The number of Nissl bodies was
reduced in the HIE group, with this trend reversed in the PD-MSC transplantation
groups. Scale bars = 100 µm. (C) A significant increase in the number of
normal neurons was found after PD-MSC transplantation, with the P7 group showing
the best treatment effect. Data are mean
Since the number of normal neurons was notably higher in the three PD-MSC transplantation groups, we further evaluated the expression levels of apoptosis-related proteins in brain tissue, including BAX, BAD and BCL-2. Western blot analysis showed that BCL-2 expression was upregulated after PD-MSC transplantation, whereas BAD and BAX expression levels were downregulated (Fig. 4A). The BCL-2 expression level was the highest in the P7 group, suggesting that PD-MSCs showed the best anti-apoptosis effects on the 7th day after HIE injury.
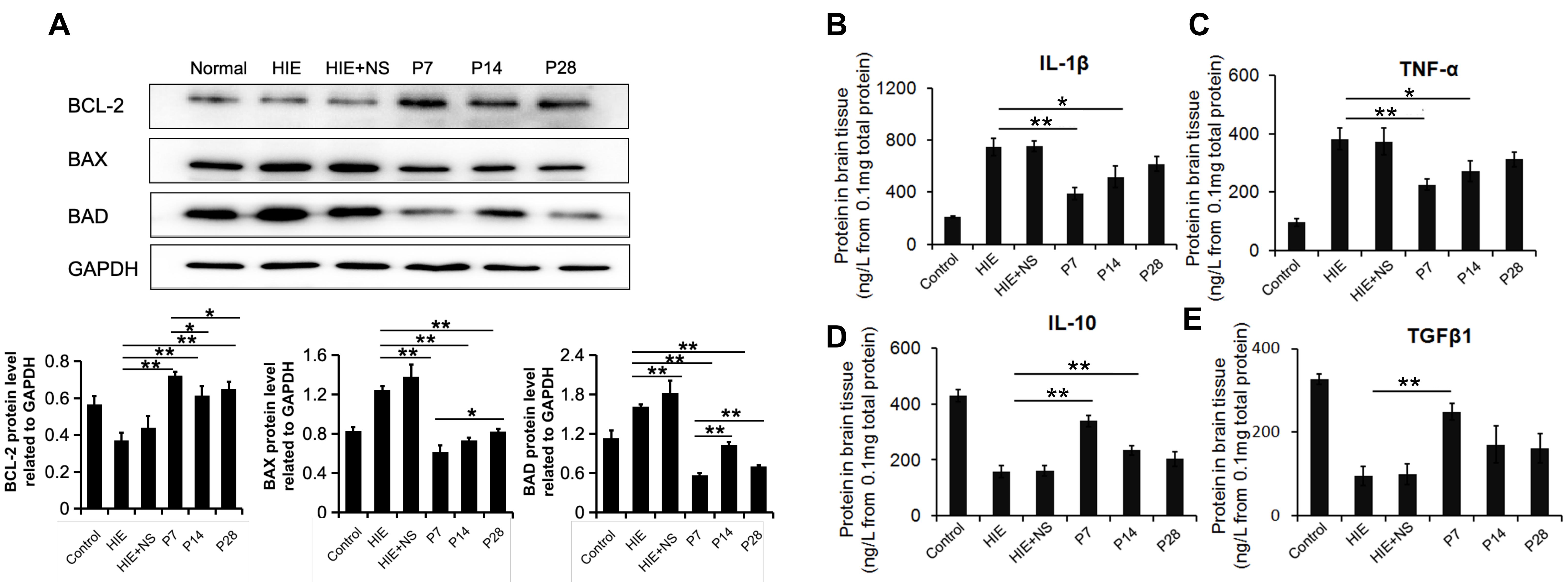
Expression of apoptosis-related proteins and inflammatory
factors. (A) The expression of apoptosis-related proteins as determined by
Western blot assay. PD-MSC transplantation increased the level of BCL-2, which
inhibits cell apoptosis. In contrast, PD-MSC transplantation decreased the levels
of BAX and BAD, which promote apoptosis. GAPDH was used as a loading control.
(B–E) Protein expression levels of IL-1
To study the inflammation processes in the HIE model, the expression levels of
anti-inflammatory cytokines were also examined. PD-MSC transplantation reduced
production of the proinflammatory cytokines IL-1
We next conducted flow cytometry with annexin V/propidium iodide (PI) to
investigate the effects of PD-MSCs on the apoptosis of injured neurons. As shown
in Fig. 5A, approximately 8% of neurons were apoptotic (p
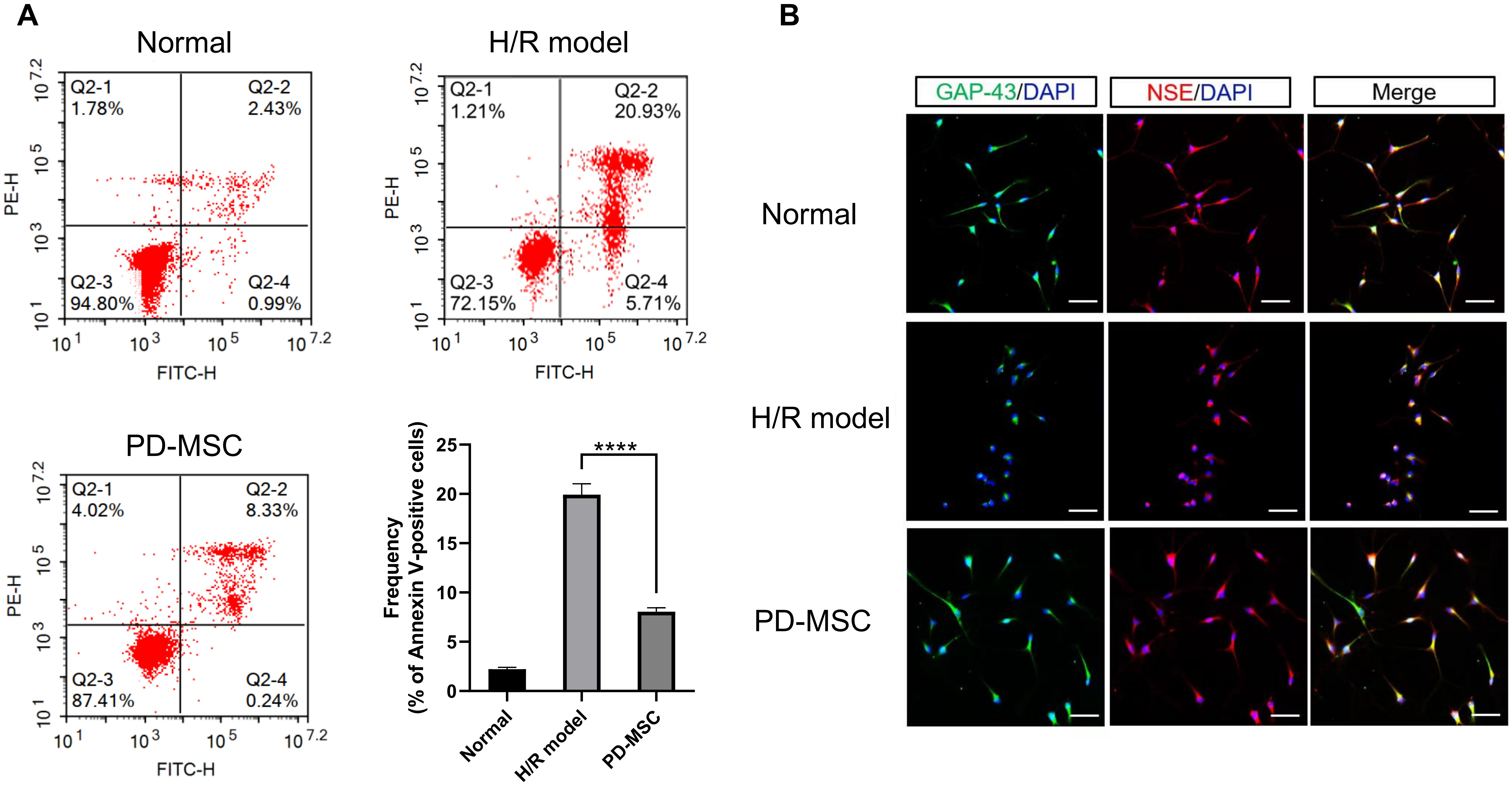
Apoptosis was inhibited by PD-MSCs in vitro. (A)
Annexin V-FITC/propidium iodide (PI) flow cytometry was performed to investigate
neuronal apoptosis. Results are mean
Protein extracted from the brain tissue of HIE model animals was used to
stimulate PD-MSCs. The results showed that protein stimulation significantly
upregulated the expression levels of IL-10 and TGF-
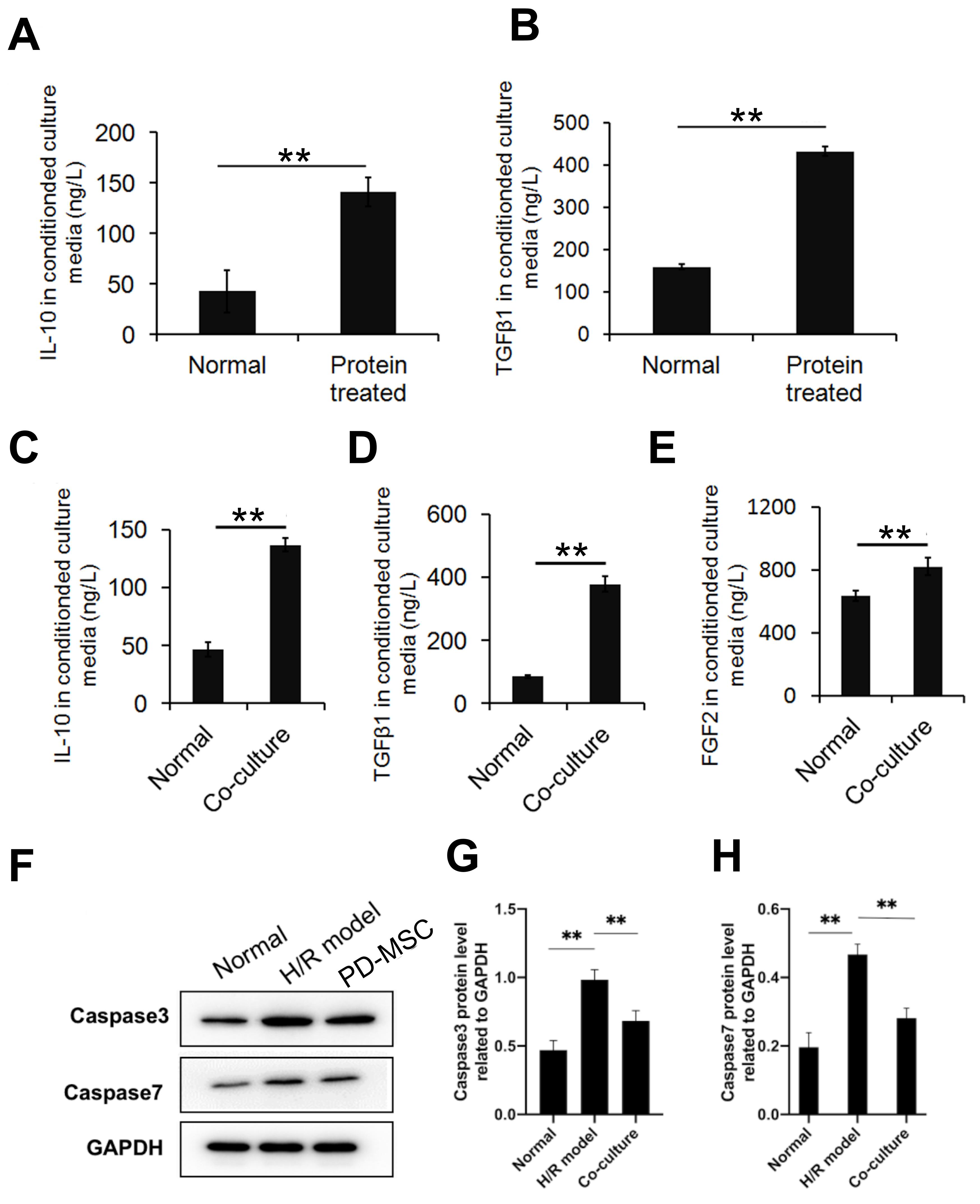
Expression levels of apoptosis-related proteins and inflammatory
factors in vitro. (A,B) Anti-inflammatory cytokines in PD-MSCs were
upregulated after stimulation with proteins from the HIE model. Results shown are
mean
HIE is a type of neonatal encephalopathy caused by systemic hypoxaemia and/or reduced cerebral blood flow resulting from an acute peripartum or intrapartum event. Researchers usually use the common carotid artery block method to develop animal models of neonatal HIE brain injury. However, this model does not accurately reflect intrauterine HI injury caused by decreased placental perfusion or disruption in the delivery of oxygen and glucose [20, 21]. Besides, the focal distribution of cerebral injuries caused by carotid artery block is more similar to a neonatal stroke rather than the deeper and widespread damage in neonatal HIE. Therefore, we developed a new type of intrauterine HI rat model by clipping the bilateral uterine arteries, which better reflects the physiological process of HIE caused by intrauterine ischaemia and hypoxia. We then conducted human PD-MSC transplantation experiments using this model, which we believe more closely reflects intrauterine HI injury. The model allowed investigation of the neuroprotective efficacy and optimal treatment timing of PD-MSCs in newborn pups with HIE.
Our results showed that PD-MSC treatment for HIE could improve growth, ameliorate behavioral function, and repair brain damage. Rats in the HIE group were insufficiently groomed and had low motor activity. This could be ameliorated by PD-MSC transplantation, which significantly reduced neuron death and improved neurological functions including motor coordination, muscle strength, and learning and memory abilities. The optimal effects of PD-MSC transplantation were observed in the P7 group, in which the rats were almost identical to normal controls in terms of weight gain, recovery of brain damage and neurological function. Ding et al. [22] reported similar results in rats suffering from HI brain injury caused by common carotid artery block. Previous studies have suggested that MSC transplantation immediately after damage, and especially before the peak of the inflammatory response, could result in better therapeutic efficacy [23, 24]. However, it is almost impossible to complete the transplantation of MSC within hours, unless these cells have been collected and prepared in advance. We found that PD-MSC transplantation 7 days after HIE insult had good therapeutic effect, and that early treatment gave better results than later transplantation. These results provide strong support for the clinical application of PD-MSC autologous reinfusion for HIE treatment.
The research focus for MSC therapeutic mechanisms has shifted from engraftment
and differentiation into neurons, to multiple functions such as reduction of
inflammation, inhibition of apoptosis, and decreased fibrosis [7, 23, 25].
Shortly after HIE damage, the primary phase of injury is caused by activation of
excitotoxicity, oxidative stress, multiple programmed cell death pathways, and
inflammatory reactions. Apoptosis has been described as an important mechanism in
both the early and chronic phases of HIE [4]. To investigate the cellular
mechanisms and potential therapeutic effects of PD-MSC transplantation for
repairing damage caused by hypoxic-ischaemic injury to the neuron in
vitro, we established the H/R cells model for further research on
anti-inflammatory and anti-apoptotic effects of PD-MSC through paracrine
pathway. In the present study, PD-MSCs were found to suppress the
inflammatory response by upregulating anti-inflammatory proteins such as
IL-1
The satisfactory therapeutic effect achieved by PD-MSCs in this intrauterine HI model hints at their potential for treating neonatal HIE. To date, several clinical trials have reported that umbilical cord blood cells and umbilical cord tissue-derived MSCs are feasible and safe for HIE treatment [29, 30, 31]. Compared to MSCs obtained from adult fat or bone marrow, MSCs derived from birth-associated gestational tissues have better reparative effects and a faster rate of cell proliferation [23]. However, since umbilical cord blood coagulates within a few minutes of delivery, it is difficult to collect sufficient amounts for MSC preparation after completing neonatal rescue. Moreover, the small number of MSCs present in umbilical cord blood and umbilical cord tissue limits their clinical application. PD-MSCs have significant advantages over other MSCs in terms of cell number, quantity and ease of access. The placenta is stable and clinicians have sufficient time to preserve the tissue and prepare it for MSC extraction. MSCs extracted from the placenta for autologous reinfusion may also prevent rejection reactions, viral infections, and ethical concerns. Although the preparation of PD-MSCs for autologous reinfusion took several days after birth, our study found that therapy started 7 days after delivery was still effective. Considering the high safety and acceptance of PD-MSC autologous transplantation, it is worthwhile collecting and preparing PD-MSCs for the supportive treatment of HIE when HI injury is suspected during delivery. Autologous reinfusion therapy with PD-MSCs may thus be a new method to treat HIE.
In summary, human PD-MSC transplantation improves neurological function in rats with intrauterine hypoxic-ischaemic encephalopathy by reducing apoptosis and inflammatory reactions. PD-MSCs showed anti-inflammatory and anti-apoptotic effects of PD-MSCs through paracrine pathway in vitro. Intravenous injection of PD-MSCs at 7, 14 and 28 days after HI damage in an intrauterine HI rat model was found to improve learning, memory and motor function by inhibiting apoptosis and inflammatory damage. PD-MSC transplantation at 7 days after HI damage showed the best effect of neuroprotection, which may be the optimal time of PD-MSC transplantation in the tertiary phase of HIE. These findings indicate the potential application of autologous reinfusion therapy with PD-MSCs for the treatment of HIE.
BAD, BCL-2-associated agonist of cell death; BAX, BCL-2-associated X protein;
BCL-2, B-cell lymphoma-2; DAPI, 4
The data that support the findings of this study are available from the corresponding author upon reasonable request.
LWY, YQZ, YMZ, YZ and RLP designed the research study. LWY, YQZ, YYX, YMZ, FH, RLP and YMH performed the research. YMZ, LWY, RLP, JRC and YMH provided help and advice on the material or patients. LWY, YQZ, YYX, RLP, JRC, FH and YMH analyzed the data. LWY, YQZ, YYX, JRC and RLP wrote the manuscript. All authors contributed to editorial changes in the manuscript. All authors read and approved the final manuscript. All authors have participated sufficiently in the work and agreed to be accountable for all aspects of the work.
The use of rats and the studies performed were carried out in accordance with the Public Health Service policy on the humane care and use of laboratory animals, and the protocol was approved by the Institutional Animal Care and Use Committee of Zhejiang Provincial People’s Hospital (ZJPPHEC 2021020). The use of human placenta was approved ty the Ethics Committee of Zhejiang Provincial People’s Hospital (ZJPPHEC 2020O055) and written informed consent was obtained from all subjects.
We are grateful for the excellent work of all of the laboratory staff. We appreciate that our patients donated their placentas for research. We appreciate Xiaozhou Mou and Jinfu Wang for his assistance in revising the manuscript.
This research was funded by the Natural Science Foundation of Zhejiang Province, grant number LGF19H040013 (Li-Wei Yang).
The authors declare no conflict of interest.
Publisher’s Note: IMR Press stays neutral with regard to jurisdictional claims in published maps and institutional affiliations.